INTRODUCTION
GaN devices continue to be a key element in many radar, electronic warfare, satellite and terrestrial communication systems. GaN devices offer a number of advantages1. For example, GaN devices have a high breakdown field due to a large bandgap that enables them to operate at higher voltages. Combining this with a high saturation velocity and corresponding large charge capability, GaN devices are ideal for high power applications. Adding excellent thermal conductivity, it is easy to see why the utilization of GaN devices continues to grow. In a recent study, Yole Développement expects the GaN industry to grow with a 23% CAGR between 2017 and 2023, driven by telecom and defense applications. 2
Some of the most popular GaN devices are wideband RF power amplifiers. Amplifiers are described by multiple characteristics including gain, frequency response or bandwidth, power output, linearity, efficiency, and noise figure. Two key characteristics that are often used to describe the quality of an amplifier are linearity and efficiency. The relative importance of the two attributes depends upon the application. For example, in a satellite-based application, efficiency may be more important as there is limited power available on a satellite. In terrestrial wireless communications, the relative importance may be more equal. Communication systems, such as those based on 5G standards, utilize wideband modulation schemes with significant linearity requirements. In addition, because of the amount of base stations needed to support the systems, it requires acute attention to power efficiency to manage operating expenses. Unfortunately, the power levels to maintain amplifier linearity are often well below the drive levels needed for maximum efficiency.
LINEARITY vs EFFICIENCY
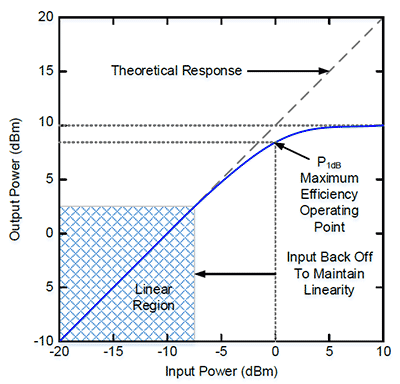
This article will focus on the applications in which amplifier linearity is the critical attribute. In Figure 1, the blue line illustrates typical amplifier behavior. Linearity is measured by increasing the input power and observing the output power until the amplifier enters compression. Often amplifier linearity is specified at the input power level at which corresponding output power is 1 dB lower than the theoretical linear response, P1dB, which has been historically considered the point at which amplifiers operate most efficiently.
With the simultaneous need for linearity and efficiency, it is crucial to optimize the input back off (IBO) value. Too much back-off sacrifices efficiency and causes the amplifier to be oversized and costlier (to reach the required output power), while too little brings increasing compression and signal degradation. Therefore, measuring amplifier linearity accurately under realistic operating conditions is of considerable value to GaN engineers.
LINEARITY ASSESSMENT ALTERNATIVES
There are a number of alternatives for measuring and expressing amplifier linearity. Three common methods are: 1) characterization of amplifier intermodulation distortion (IMD), 2) noise power ratio (NPR) measurements, and 3) crest factor (CF), or peak-to-average power ratio (PAPR) measurements.
LINEARITY ASSESSMENT TOOL COMPARISON
The linearity of an amplifier was measured with all three approaches. Using a Noisecom noise source or a two-tone source, a signal was applied to the amplifier starting from -25 and increased in one dB steps until reaching -15 dBm. The results for each measurement are shown below. Reductions in gain, crest factor, NPR, and the delta between intermodulation products and the carrier signals all indicate an amplifier is behaving non-linearly.
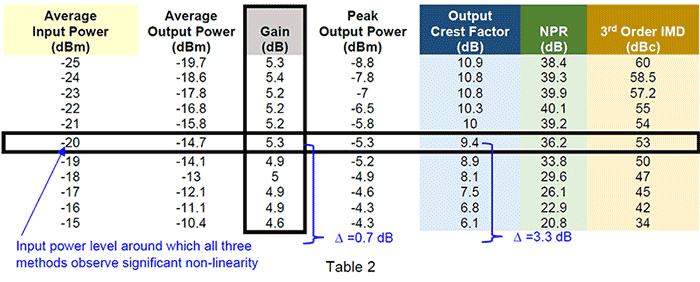
All three measurements show that as the power increases, the amplifier is compressing the signal and operating non-linearly. Moreover, all three measurements indicate the amplifier starts compressing when the average input power is around -20 dBm. However, only the crest factor method clearly reveals the amount of compression. It should be noted that although there is a reduction in gain around -20 dBm, even at -15 dBm, the gain is only reduced less than 1 dB. In contrast, the other linearity measurements show much more significant compression for the peak power. For example, the crest factor decreases by more than 3 dB. Compression measurements using average power alone are not sufficient to identify significant impairments for signals with high crest factors; e.g., OFDM signals used in 5G and Wi-Fi communication systems.
When comparing the three linearity measurement approaches, there are clear advantages to the crest factor approach.
Clearer indication of meaningful signal compression.
Conventional, average power-only, compression measurements will under-report the degree of signal impairment that will occur for wideband communication signals.Lower cost.
The crest factor approach utilizes low cost noise sources and wideband USB peak power sensors. Spectrum analyzers can be multiples of the price of a USB sensor. An analog signal generator also adds significant expense.Simpler, less error prone measurements.
Spectrum analyzers can be complex to configure and present difficulty with interpreting results.Higher accuracy.
The uncertainty for a power sensor measurement is usually in the tenths of a dB, while spectrum analyzers and signal generators are typically in the 1-2 dB range.
Utilizing the complementary cumulative distribution function (CCDF) of the crest factor adds the extra element of probability of occurrence. Crest factor measurements provide a single value based on a calculation from the highest single peak power value in a population of measurement samples. However, if this peak only occurs once out of a million samples, it may not present a problem during actual use of the amplifier. Quantifying the degree of peak impairment using CCDF can provide the value of crest factor which occurs with a specific probability (e.g., 0.1% or one in 1000 samples), which can be helpful when considering bit-error-rate (BER) or error vector magnitude (EVM) effects of amplifier compression.
Next, the three linearity assessment tools will be examined more closely to determine where the benefits of the crest factor approach are derived.
IMD / 3rd ORDER INTERCEPT
When an amplifier goes into compression, it becomes non-linear and produces signal harmonics. These harmonics can mix together and result in intermodulation products. The second, third, and higher order harmonics are usually outside of the amplifier bandwidth and are easy to filter out. However, some of the intermodulation products are close to the signals intended to be amplified and can cause intermodulation distortion (IMD). Figure 2 shows the intermodulation products for two equal amplitude tones (A and B).
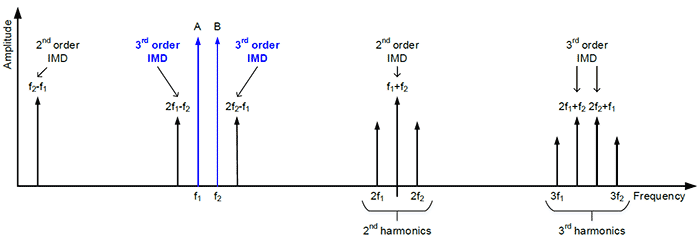
Figure 3
Because of their proximity to the intended signals, amplifier manufacturers are concerned with the amplitude of the 3rd order products and often specify their IP3 rating or a 3rd order intercept value. To determine this value, the amplitude of the 3rd order products are plotted in a similar way as shown in Figure 1. Note: these products increase at a rate of three times that of the desired signal. The intersection of the theoretical extensions of these lines is called the 3rd order intercept point, which can be denoted as TOI or IP3. The higher the IP3 value the better the linearity and the lower the IMD.
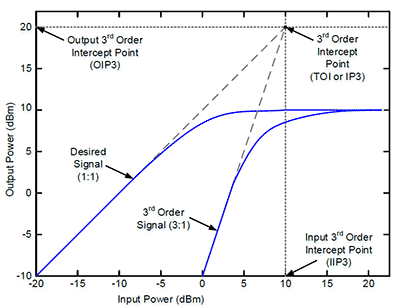
To create this plot, a signal generator or generators are used to create the two tones and a spectrum analyzer or vector network analyzer (VNA) is used measure the desired signal and 3rd order products. A disadvantage of this approach is that the measurement is only conducted using two tones. In practice, the signal provided to the amplifier often has significantly more tones. For example, an LTE-A (5 CCs) signal has 6000 effective sub-carriers or tones and a 5G (NR) signal may have 3300. Two continuous wave (CW) tones do not represent the dynamic loading that an amplifier would experience in actual operation. The crest factor is only 3 dB for a two-tone signal yet can approach 15 dB for a 5G signal, so the power supply and thermal effects could be much different with increasing power of just two tones rather than they would be for a high-count multi-tone or noise-like signal. Another consideration is the phase coherency between tones. If they are random in phase, the measurement may be different from phase coherent tones. This is further complicated in the case of LTE or 5G signals, where the physical layer is based on the orthogonality of the carriers/tones.
NOISE POWER RATIO
Another approach for quantifying amplifier linearity is to determine its noise power ratio (NPR). In this approach, white noise is used to simulate a multitone/carrier signal. An additive white gaussian noise (AWGN) signal, such as those provided by companies like Noisecom, has a high CF and represents a wideband communication signal much better than a two-tone IMD test stimulus. The noise is bandlimited by a filter to either the useful bandwidth of the amplifier or the bandwidth of the expected signal the amplifier will receive. The resultant signal is then passed through a notch filter (typically > 50 dB down from the pass band amplitude) with a width of about 1 percent or less of the filtered noise bandwidth3. When this signal is applied to the amplifier, the amplitude is increased to determine when it behaves non-linearly. As discussed above, in the non-linear region, the tones will mix to create intermodulation products. Because the noise signal is equivalent to a large number of tones, the individual IMD products cannot be easily measured. Instead the aggregate power of the IMD products at the notch frequency is observed. As the products increase, the effective depth of the notch will decrease. In Figure 4, the blue signal is the input signal to the amplifier with a notch depth of ~40 dB. The black signal is the amplified signal. As can be seen, the depth of the notch has decreased substantially – signifying the amplifier is in compression. Similar to two-tone IMD testing, NPR is typically measured with a spectrum analyzer or VNA. An added expense is a high-quality filter with sufficient notch depth to observe the IMD products of interest.
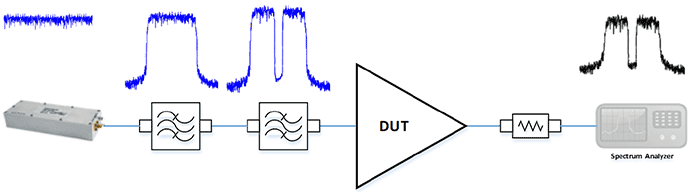
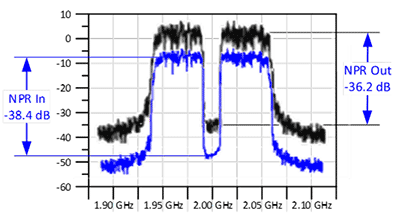
A third approach for characterizing an amplifier’s linearity is with a crest factor measurement. Crest factor is the ratio of peak to average power. Similar to the NPR measurement, the amplifier is provided a bandlimited noise signal to stimulate it with a signal more closely approximating what an amplifier would experience in actual operation. Using a directional coupler or signal splitter, the incident signal is measured with a wideband peak power sensor. The video bandwidth of the sensor (as well as the coupler or splitter) should be at least as wide as the bandwidth of the noise signal. Otherwise, the sensor measurement will be distorted. Figure 5 shows two power measurements. The blue trace is the measurement with a sensor of sufficient video bandwidth. The red trace shows the impact of insufficient bandwidth.
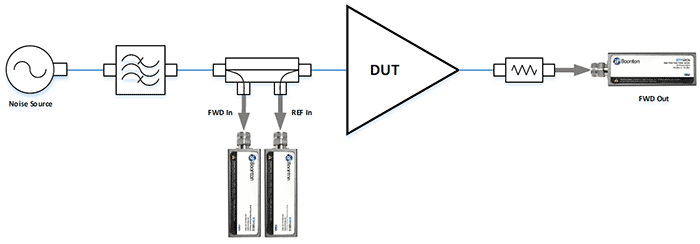
Figure 6
A second measurement is made at the output of the amplifier (attenuation may be needed to keep the signal in the measurement range of the sensor). The crest factors of the input and output signals are compared. If the output crest factor is less, then the amplifier is compressing the signal’s highest peaks. In addition, by comparing the input and output average power, the amplifier gain can be determined. For the most accurate measurement, a third sensor can be used to determine if a portion of the input signal is reflected rather than amplified. Figure 6 shows the corresponding test setup. Note: just monitoring the gain changes with average power measurements does not give a complete description of the amplifier linearity. An average power gain reduction can be significantly smaller than the compression observed by a multi-tone signal. This will be illustrated later in this article.
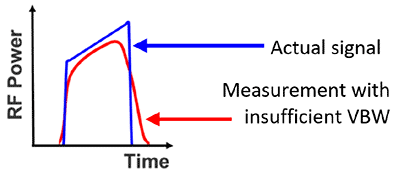
COMPLEMENTARY CUMULATIVE DISTRIBUTION FUNCTION
To get additional insight into the amplifier performance, a CCDF can be used. A CCDF curve shows the amount of time a signal spends above the average power level of the measured signal at each power level relative to the average. Or equivalently, the CCDF curve displays the probability that the signal power will be above the average power at each power level. With an average input power level of -12.7 dBm, the amplifier under test displays a crest factor of approximately 9 dB at the input (CH1) and the output (CH2) 0.01% of the time – Figure 7.
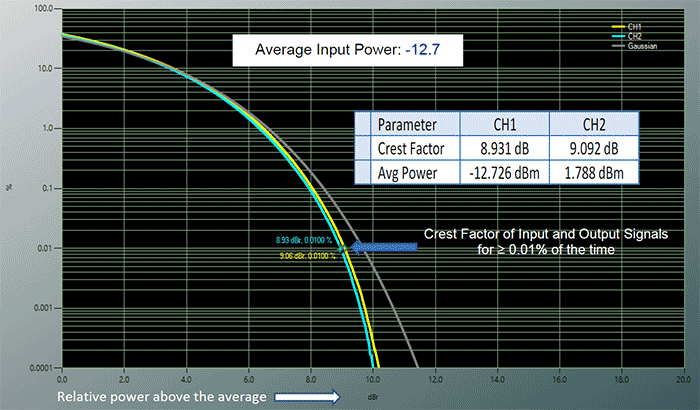
Figure 8
However, as the power is increased by 10 dB to -2.5 dBm, the crest factor at the output has degraded significantly to 5.4 dB – indicating a substantial degree of amplifier compression; see Figure 8.
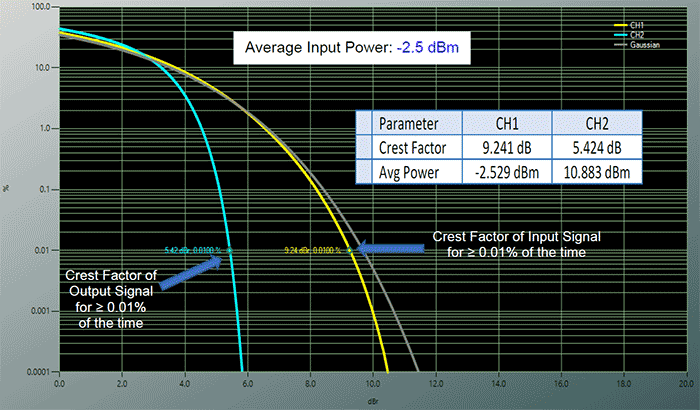
Figure 9
REAL WORLD CREST FACTOR ASSESSMENT EXAMPLE
The additional information provided by using crest factor and associated CCDF has shed light on the applicability of using classes of amplifierREAL WORLD CREST FACTOR ASSESSMENT EXAMPLEs previously thought to be unacceptable for communications related applications. For example, the Empower RF Systems model 2223 utilizes GaN on SiC in a class AB broadband high-power amplifier (HPA). It offers a wide frequency response (500 to 6000 MHz), high gain (53 dB) and a 150 W minimum output power. Its class AB design offers compact size and very high efficiency given its bandwidth. This amplifier exemplifies a multi-mode amplifier – an amplifier capable of efficient brute force power and linear performance for communications or product testing. Using traditional methods for measuring linearity will falsely characterize its linearity and suitability for transmitting digitally modulated waveforms.
Two linearity test methods were performed on the model 2223 to determine P1dB. The first method utilizes a continuous wave (CW) signal where the input power level is increased until 1 dB compression is observed. This was done at multiple frequencies across the band and the results are shown in Table 2. The second method utilizes the Crest Factor method for determining P1dB. In this case, the amplifier is provided a 64QAM signal having a 6.3 dB crest factor. To determine the 1 dB compression point using crest factor method and CCDF, the amplifier is operated at each of the test frequencies and the input power is increased until the measured output crest factor decreases 1 dB to 5.3 dB.
Frequency (MHz) |
P1dB CW (dBm) |
P1dB CF (dBm) |
---|---|---|
500 | 48.5 | 50.5 |
1000 | 49 | 51.6 |
1500 | 49 | 51.7 |
2000 | 47.9 | 51.8 |
2500 | 48.2 | 51.6 |
3000 | 46.5 | 49.1 |
3500 | 44.8 | 47.8 |
4000 | 46 | 47.2 |
4500 | 46.4 | 47.7 |
5000 | 47 | 48.4 |
5500 | 45.5 | 45.7 |
6000 | 45.8 | 45.1 |
Table 3
Table 2 shows that on average the CW method indicates the P1dB compression point is about 2 dB below the P1dB when measured with CF. The reason for the difference is transistor gain versus temperature variation. In a class AB amplifier, the transistor consumes more power as output power is increased. As the junction temperature increases, the transistor gain decreases. In a class A amplifier, one would see the opposite effect. In most GaN HPAs, the gain will vary -0.012 dB/°C (junction temperature) per stage of amplification.
For example, consider a Class A amplifier stimulated with a CW signal. When the output power is low, the transistors are actually consuming the most power and junction temperatures are near their maximum for the specific operating condition. As the output power is increased, the junction temperature of the transistor is reduced. This results in gain expansion extending the “apparent” compression point. However, when the same amplifier is stimulated with a modulated signal with a high CF, the modulation does not exhibit the gain expansion since the junction temperatures correlate to the average power and therefore remains high. This further highlights the necessity to stimulate an amplifier with a signal representing the signal with which it is intended to be used. To examine the phenomena above in more detail, in a linear power amplifier (PA), the output power can be described by the equation Pout(ωt)= α + G x f(ωt), where f(ωt) is the input signal, α is a constant, and G is the gain of the amplifier. G can also vary with temperature as described by the equation (-0.012 dB/°C) x (Thermal Resistance) of the junction (°C/W) x (Power Dissipated). In a class AB amplifier, if the thermal resistance is 1°C/W and the power dissipation from small signal to large signal increases by 50W, the equation will show a gain reduction of -0.6 dB solely due to the increase in junction temperature. Conversely, when operating the same device in class A configuration, the power dissipated will decrease since part of the power will now go to the load. Using the same gain equation, the resultant gain will now increase because the power dissipated is reduced. In both cases, this gain variation has no relation to the true linearity of the PA (the linearity that really changes the integrity of the input signal). True linearity is assessed by measuring compression of the signal peaks relative to the average power.
CONCLUSION
Utilization of GaN technology will accelerate in coming years, driven primarily by advances in commercial and military radar as well as the build out of 5G networks. 5G has very demanding linearity requirements. Traditional tools for assessing amplifier linearity used in the networks are no longer sufficient to predict real-world performance. When compared to traditional tools, analyzing crest factor reduction caused by amplifier compression combined with the statistical analysis offered by CCDFs offers a clearer and more true indication of signal compression, a lower cost alternative, simpler, less error prone measurements, and higher accuracy. In addition, this tool shows how amplifiers previously considered not suitable for communications applications actually can be good alternatives to traditionally used amplifiers.
1 Moore, Andrew and Jimenez, Jose. GaN RF Technology for Dummies®, Qorvo Special Edition. John Wiley & Sons, Inc., 2015.
2 RF GaN market: applications, players, technology, and substrates 2018-2023 report, Yole Développement, 2018.
3 Katz, Allen and Gray, Robert. Noise Power Ratio Tutorial. Linearizer Technology.